Abstract
Graphitic electrode is commonly used in electrochemical reactions owing to its excellent in-plane conductivity, structural robustness and cost efficiency1,2. It serves as prime electrocatalyst support as well as a layered intercalation matrix2,3, with wide applications in energy conversion and storage1,4. Being the two-dimensional building block of graphite, graphene shares similar chemical properties with graphite1,2, and its unique physical and chemical properties offer more varieties and tunability for developing state-of-the-art graphitic devices5,6,7. Hence it serves as an ideal platform to investigate the microscopic structure and reaction kinetics at the graphitic-electrode interfaces. Unfortunately, graphene is susceptible to various extrinsic factors, such as substrate effect8,9,10, causing much confusion and controversy7,8,10,11. Hereby we have obtained centimetre-sized substrate-free monolayer graphene suspended on aqueous electrolyte surface with gate tunability. Using sum-frequency spectroscopy, here we show the structural evolution versus the gate voltage at the graphene–water interface. The hydrogen-bond network of water in the Stern layer is barely changed within the water-electrolysis window but undergoes notable change when switching on the electrochemical reactions. The dangling O–H bond protruding at the graphene–water interface disappears at the onset of the hydrogen evolution reaction, signifying a marked structural change on the topmost layer owing to excess intermediate species next to the electrode. The large-size suspended pristine graphene offers a new platform to unravel the microscopic processes at the graphitic-electrode interfaces.
This is a preview of subscription content, access via your institution
Access options
Access Nature and 54 other Nature Portfolio journals
Get Nature+, our best-value online-access subscription
24,99 € / 30 days
cancel any time
Subscribe to this journal
Receive 51 print issues and online access
199,00 € per year
only 3,90 € per issue
Rent or buy this article
Prices vary by article type
from$1.95
to$39.95
Prices may be subject to local taxes which are calculated during checkout
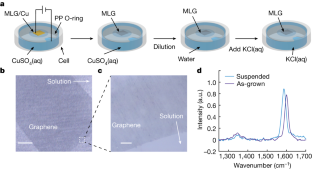
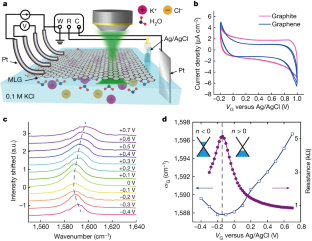

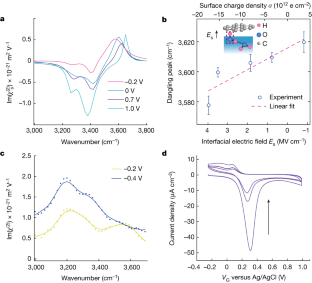
Data availability
The authors declare that the data supporting the findings of this study are available in the paper and source data files. Should any raw data files be needed in another format, they are available from the corresponding author on reasonable request. Source data are provided with this paper.
References
-
Das, R. K. et al. Extraordinary hydrogen evolution and oxidation reaction activity from carbon nanotubes and graphitic carbons. ACS Nano 8, 8447–8456 (2014).
-
Murthy, A. P., Madhavan, J. & Murugan, K. Recent advances in hydrogen evolution reaction catalysts on carbon/carbon-based supports in acid media. J. Power Sources 398, 9–26 (2018).
-
Enoki, T., Suzuki, M. & Endo, M. Graphite Intercalation Compounds and Applications (Oxford Univ. Press, 2003).
-
Bonaccorso, F. et al. Graphene, related two-dimensional crystals, and hybrid systems for energy conversion and storage. Science 347, 1246501 (2015).
-
Bie, Y.-Q. et al. Vibrational spectroscopy at electrolyte/electrode interfaces with graphene gratings. Nat. Commun. 6, 7593 (2015).
-
Peng, Q., Chen, J., Ji, H., Morita, A. & Ye, S. Origin of the overpotential for the oxygen evolution reaction on a well-defined graphene electrode probed by in situ sum frequency generation vibrational spectroscopy. J. Am. Chem. Sci. 140, 15568–15571 (2018).
-
Montenegro, A. et al. Asymmetric response of interfacial water to applied electric fields. Nature 594, 62–65 (2021).
-
Rafiee, J. et al. Wetting transparency of graphene. Nat. Mater. 11, 217–222 (2012).
-
Achtyl, J. L. et al. Aqueous proton transfer across single-layer graphene. Nat. Commun. 6, 6539 (2015).
-
Dreier, L. B. et al. Surface-specific spectroscopy of water at a potentiostatically controlled supported graphene monolayer. J. Phys. Chem. C 123, 24031–24038 (2019).
-
Ohto, T., Tada, H. & Nagata, Y. Structure and dynamics of water at water–graphene and water–hexagonal boron-nitride sheet interfaces revealed by ab initio sum-frequency generation spectroscopy. Phys. Chem. Chem. Phys. 20, 12979–12985 (2018).
-
Zhang, Y., de Aguiar, H. B., Hynes, J. T. & Laage, D. Water structure, dynamics, and sum-frequency generation spectra at electrified graphene interfaces. J. Phys. Chem. Lett. 11, 624–631 (2020).
-
Singla, S. et al. Insight on structure of water and ice next to graphene using surface-sensitive spectroscopy. ACS Nano 11, 4899–4906 (2017).
-
He, Q. et al. Electrochemical storage of atomic hydrogen on single layer graphene. J. Am. Chem. Soc. 143, 18419–18425 (2021).
-
Beams, R., Cançado, L. G. & Novotny, L. Raman characterization of defects and dopants in graphene. J. Phys. Condens. Matter 27, 083002 (2015).
-
Chen, F., Qing, Q., Xia, J., Li, J. & Tao, N. Electrochemical gate-controlled charge transport in graphene in ionic liquid and aqueous solution. J. Am. Chem. Soc. 131, 9908–9909 (2009).
-
Bard, A. J. & Faulkner, L. R. Electrochemical Methods: Fundamentals and Applications (Wiley, 2001).
-
Das, A. et al. Monitoring dopants by Raman scattering in an electrochemically top-gated graphene transistor. Nat. Nanotechnol. 3, 210–215 (2008).
-
Döppenschmidt, A. & Butt, H.-J. Measuring electrostatic double-layer forces on HOPG at high surface potentials. Colloids Surf. A 149, 145–150 (1999).
-
Sun, S. et al. Phase reference in phase-sensitive sum-frequency vibrational spectroscopy. J. Chem. Phys. 144, 244711 (2016).
-
Ji, N., Ostroverkhov, V., Tian, C. & Shen, Y. Characterization of vibrational resonances of water-vapor interfaces by phase-sensitive sum-frequency spectroscopy. Phys. Rev. Lett. 100, 096102 (2008).
-
Wen, Y.-C. et al. Unveiling microscopic structures of charged water interfaces by surface-specific vibrational spectroscopy. Phys. Rev. Lett. 116, 016101 (2016).
-
Cheng, J., Vermeulen, N. & Sipe, J. Second order optical nonlinearity of graphene due to electric quadrupole and magnetic dipole effects. Sci. Rep. 7, 43843 (2017).
-
Morita, A. Theory of Sum Frequency Generation Spectroscopy 201–218 (Springer, 2018).
-
Somorjai, G. A. & Li, Y. Introduction to Surface Chemistry and Catalysis (Wiley, 2010).
-
Zhang, Y. et al. Doping-induced second-harmonic generation in centrosymmetric graphene from quadrupole response. Phys. Rev. Lett. 122, 047401 (2019).
-
Shin, S., Kang, H., Cho, D., Lee, J. Y. & Kang, H. Effect of electric field on condensed-phase molecular systems. II. Stark effect on the hydroxyl stretch vibration of ice. J. Phys. Chem. C 119, 15596–15603 (2015).
-
Tomlinson-Phillips, J. et al. Structure and dynamics of water dangling OH bonds in hydrophobic hydration shells. Comparison of simulation and experiment. J. Phys. Chem. A 115, 6177–6183 (2011).
-
Kronberg, R., Lappalainen, H. & Laasonen, K. Revisiting the Volmer–Heyrovský mechanism of hydrogen evolution on a nitrogen doped carbon nanotube: constrained molecular dynamics versus the nudged elastic band method. Phys. Chem. Chem. Phys. 22, 10536–10549 (2020).
-
Wilhelm, F., Schmickler, W. & Spohr, E. Proton transfer to charged platinum electrodes. A molecular dynamics trajectory study. J. Phys. Condens. Matter 22, 175001 (2010).
-
Kibsgaard, J. et al. Cluster–support interactions and morphology of MoS2 nanoclusters in a graphite-supported hydrotreating model catalyst. J. Am. Chem. Soc. 128, 13950–13958 (2006).
-
Zhao, G. & Liu, G. Electrochemical deposition of gold nanoparticles on reduced graphene oxide by fast scan cyclic voltammetry for the sensitive determination of As(III). Nanomaterials 9, 41 (2018).
-
Brown, M. A., Goel, A. & Abbas, Z. Effect of electrolyte concentration on the Stern layer thickness at a charged interface. Angew. Chem. Int. Ed. 128, 3854–3858 (2016).
-
Cole, D. J., Ang, P. K. & Loh, K. P. Ion adsorption at the graphene/electrolyte interface. J. Phys. Chem. Lett. 2, 1799–1803 (2011).
-
Elliott, J., Papaderakis, A. A., Dryfe, R. & Carbone, P. The electrochemical double layer at the graphene/aqueous electrolyte interface: what we can learn from simulations, experiments, and theory. J. Mater. Chem. C 10, 15225–15262 (2022).
-
Fumagalli, L. et al. Anomalously low dielectric constant of confined water. Science 360, 1339–1342 (2018).
-
Chiang, K.-Y. et al. The dielectric function profile across the water interface through surface-specific vibrational spectroscopy and simulations. Proc. Natl Acad. Sci. USA 119, e2204156119 (2022).
-
Rezaei, M. et al. Interfacial, electroviscous, and nonlinear dielectric effects on electrokinetics at highly charged surfaces. J. Phys. Chem. B 125, 4767–4778 (2021).
-
Wen, Y.-C., Zha, S., Tian, C. & Shen, Y. R. Surface pH and ion affinity at the alcohol-monolayer/water interface studied by sum-frequency spectroscopy. J. Phys. Chem. C 120, 15224–15229 (2016).
-
Ohno, P. E., Saslow, S. A., Wang, H.-f., Geiger, F. M. & Eisenthal, K. B. Phase-referenced nonlinear spectroscopy of the α-quartz/water interface. Nat. Commun. 7, 13587 (2016).
-
Zhang, L., Tian, C., Waychunas, G. A. & Shen, Y. R. Structures and charging of α-alumina (0001)/water interfaces studied by sum-frequency vibrational spectroscopy. J. Am. Chem. Soc. 130, 7686–7694 (2008).
-
Fitts, J. P., Shang, X., Flynn, G. W., Heinz, T. F. & Eisenthal, K. B. Electrostatic surface charge at aqueous/α-Al2O3 single-crystal interfaces as probed by optical second-harmonic generation. J. Phys. Chem. B 109, 7981–7986 (2005).
-
Yang, S. et al. Stabilization of hydroxide ions at the interface of a hydrophobic monolayer on water via reduced proton transfer. Phys. Rev. Lett. 125, 156803 (2020).
-
Döppenschmidt, A. & Butt, H.-J. Measuring electrostatic double-layer forces on HOPG at high surface potentials. Colloids Surf. A 149, 145–150 (1999).
Acknowledgements
This work was supported by the National Natural Science Foundation of China (12125403, 11874123, 12221004, 12293053) and the National Key Research and Development Program of China (2021YFA1400202, 2021YFA1400503). We thank K. Liu and Carbon Six Co. for discussions on the CVD graphene growth and Y.-D. Su for discussions on the analysis of data.
Author information
Authors and Affiliations
Contributions
Y.X. and C.-S.T. devised the project. Y.X., Y.-B.M., S.-S.Y. and F.G. carried out the experiments. Y.X., F.G. and C.-S.T. analysed the data. Y.X., F.G. and C.-S.T. drafted the manuscript and all authors contributed to the final version.
Corresponding author
Ethics declarations
Competing interests
The authors declare no competing interests.
Peer review
Peer review information
Nature thanks the anonymous reviewers for their contribution to the peer review of this work.
Additional information
Publisher’s note Springer Nature remains neutral with regard to jurisdictional claims in published maps and institutional affiliations.
Extended data figures and tables
Extended Data Fig. 1 Dilution process.
Flow chart of diluting the electrolyte (CuSO4, 0.3 M) used for electrochemical etching of copper.
Extended Data Fig. 2 Equivalent circuit model of the interface.
The equivalent circuit and illustration of the EDL at the gated MLG–water interface.
Extended Data Fig. 3 MLG carrier density and Fermi level.
Carrier density n (a) and the Fermi level μ (b) of graphene versus VG.
Extended Data Fig. 4 Supplementary Raman spectra.
a–c, Raman spectrum of the monolayer graphene sample at different gate voltage VG.
Extended Data Fig. 5 Sketch of the PS SFVS setup.
Collinear geometry used in the PS SFVS detection.
a, Im(χ(2)) spectra at different VG. Dashed curves are the calculated Im(\({\chi }_}^{(2)}\)) spectra of graphene with Vpzc = 0.5 V. b, Im[\({\chi }_}^{(2)}\)(ωIR = 3,000 cm−1) + χ(3)(ωIR = 3,000 cm−1)Ψ] versus VG. The shaded region denotes uncertainty. Error bars are calculated from 30 averages.
Source data
Rights and permissions
Springer Nature or its licensor (e.g. a society or other partner) holds exclusive rights to this article under a publishing agreement with the author(s) or other rightsholder(s); author self-archiving of the accepted manuscript version of this article is solely governed by the terms of such publishing agreement and applicable law.
About this article
Cite this article
Xu, Y., Ma, YB., Gu, F. et al. Structure evolution at the gate-tunable suspended graphene–water interface. Nature (2023). https://ift.tt/G9BXhQR
-
Received:
-
Accepted:
-
Published:
-
DOI: https://ift.tt/G9BXhQR
Comments
By submitting a comment you agree to abide by our Terms and Community Guidelines. If you find something abusive or that does not comply with our terms or guidelines please flag it as inappropriate.
"interface" - Google News
August 30, 2023 at 10:05PM
https://ift.tt/ZLshSlR
Structure evolution at the gate-tunable suspended graphene–water interface - Nature.com
"interface" - Google News
https://ift.tt/1GFviWr
https://ift.tt/AY9oPSa
Bagikan Berita Ini
0 Response to "Structure evolution at the gate-tunable suspended graphene–water interface - Nature.com"
Post a Comment